Particle accelerator
The basic principle behind the particle accelerator is simple: Collide things together at high energy and detect what comes out.
In 1909, Ernest Rutherford discovered that the atom consists of a tiny, massive, positively charged nucleus surrounded by a billowy cloud of light electrons 10,000 times as large. To understand the structure of this atomic nucleus, scientists have developed various "probes" in the years sinceâthe most useful being the electrically neutral neutron and a variety of electrically charged particles. As the neutron is not repelled by the nuclear charge, low-velocity ones do fine as probes (see nuclear fission). Charged particles, however, penetrate best when they are highly energetic. Pumping up the energy of such probes is the role of the particle accelerator.
The very first high-energy probes were provided by nature, in terms of the alpha, beta, and gamma rays of radioactive elements. In fact, Rutherford used the high-energy alphas from radium as a probe to establish his model of the atom. Although cosmic rays have been (and still are) used as probesâthe positron was discovered in this wayâalmost all the advances in particle physics have been made using man-made accelerators with ever-increasing power.
As the power of the probes increased, a plethora of particles were discovered, developing into what was called a "particle zoo." Eventually, they were all organized according to a system called the Standard Model. In the atom bomb, matter is turned into energy; in a high-energy particle accelerator, energy is turned into matter.
A particle accelerator uses electric fields to propel electrically charged particles to high speeds and to contain them. An ordinary CRT television set is a simple form of an accelerator.
There are two basic types of accelerators: linear and circular. Both designs have limitations. The longer a linear accelerator is, the higher the energy that can be imparted, so the limits are set by the practical length. In a circular design, the length is unbounded. The limits here arise because making electrical charges go in circles causes them to shed energy. As they speed up, more energy is shed, until eventually they shed energy just as quickly as it can be pumped in.
Linear accelerators
In a linear accelerator (linac), particles are accelerated in a straight line with a target of interest at one end.
Linear high-energy accelerators use a linear array of plates (or drift tubes) to which an alternating high-energy field is applied. As the particles approach a plate, they are accelerated towards it by an opposite polarity charge applied to the plate. As they pass through a hole in the plate, the polarity is switched so that the plate now repels them and they are then accelerated by it towards the next plate. Normally a stream of "bunches" of particles are accelerated, so a carefully controlled AC voltage is applied to each plate to continuously repeat this for each bunch.
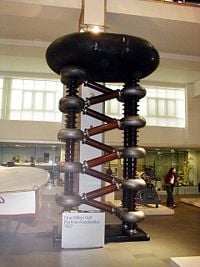
As the particles approach the speed of light, the switching rate of the electric fields becomes so high that they operate at microwave frequencies, and so RF cavity resonators are used in higher energy machines instead of simple plates.
DC accelerator types capable of accelerating particles to speeds sufficient to cause nuclear reactions are Cockcroft-Walton generators, or voltage multipliers, which convert AC to high voltage DC, or Van de Graaff generators that use static electricity carried by belts.
The largest and most powerful particle accelerators, such as the RHIC, the LHC (scheduled to start operation in 2008) and the Tevatron, are used for experimental particle physics.
Particle accelerators can also produce proton beams, which can produce "proton-heavy" research or medical isotopes, as opposed to the "neutron-heavy" ones made in fission reactors. An example of this type of machine is LANSCE at Los Alamos.
Examples
Everyday examples of particle accelerators are those found in television sets and X-ray generators. Low-energy accelerators, such as cathode ray tubes and X-ray generators, use a single pair of electrodes with a DC voltage of a few thousand volts between them. In an X-ray generator, the target itself is one of the electrodes. A low-energy particle accelerator, called an ion implanter, is used in the manufacture of integrated circuits.
Linacs are very widely used. They are also used to provide an initial low-energy kick to particles before they are injected into circular accelerators. The longest linac in the world is the Stanford Linear Accelerator, SLAC, which is 3 km (2 miles) long. SLAC is an electron-positron collider.
Linear accelerators are also widely used in medicine, for radiotherapy and radiosurgery. Medical grade Linacs accelerate electrons using a klystron and a complex bending magnet arrangement, which produces a beam of 6-30 million electron-volt (MeV) energy. The electrons can be used directly or they can be collided with a target to produce a beam of X-rays. The reliability, flexibility, and accuracy of the radiation beam produced has largely supplanted the older use of Cobalt-60 therapy as a treatment tool.
Tandem electrostatic accelerators
In a tandem accelerator, the negatively charged ion gains energy by attraction to the very high positive voltage at the geometric center of the pressure vessel. When it arrives at the center region known as the high voltage terminal, some electrons are stripped from the ion. The ion then becomes positive and accelerated away by the high positive voltage. Thus, this type of accelerator is called a "tandem" accelerator. The accelerator has two stages of acceleration, first pulling and then pushing the charged particles. An example of a tandem accelerator is ANTARES (Australian National Tandem Accelerator for Applied Research).
Circular accelerators

In the circular accelerator, particles move in a circle until they reach sufficient energy. The particle track is typically bent into a circle using electromagnets. The advantage of circular accelerators over linear accelerators is that the ring topology allows continuous acceleration, as the particle can transit indefinitely. Another advantage is that a circular accelerator is relatively smaller than a linear accelerator of comparable power (i.e. a linac would have to be extremely long to have the equivalent power of a circular accelerator).
Depending on the energy and the particle being accelerated, circular accelerators suffer a disadvantage in that the particles emit synchrotron radiation. When any charged particle is accelerated, it emits both electromagnetic radiation and secondary emissions. As a particle traveling in a circle is always accelerating towards the center of the circle, it continuously radiates towards the tangent of the circle. This radiation is called synchrotron light and depends highly on the mass of the accelerating particle. For this reason, many high energy electron accelerators are linacs.
Synchrotron radiation
The shedding of energy by electrical particles forced to curve is called synchrotron radiation. The tighter the curve, the greater the energy shed, which is why circular accelerators are as large as possible, minimizing the curvature.
Some circular accelerators have been built to deliberately generate radiation (called synchrotron light) as X-rays, for example, the Diamond Light Source being built at the Rutherford Appleton Laboratory in England or the Advanced Photon Source at Argonne National Laboratory in Illinois. High-energy X-rays are useful for X-ray spectroscopy of proteins or X-ray absorption fine structure (XAFS).
Synchrotron radiation is more powerfully emitted by lighter particles, so these accelerators are invariably electron accelerators. Synchrotron radiation allows for better imaging as researched and developed at SLAC's SPEAR. In contrast, particle physicists are increasingly using more massive particles, such as protons (or nuclei), in their accelerators to get to higher energies. These particles are composites of quarks and gluons, which makes analyzing the results of their interactions much more complicated, and also of much scientific interest.
History of cyclotrons
The earliest circular accelerators were cyclotrons, invented in 1929 by Ernest O. Lawrence at the University of California, Berkeley. Cyclotrons have a single pair of hollow, D-shaped plates to accelerate the particles and a single dipole magnet to curve the track of the particles. The particles are injected in the center of the circular machine and spiral outwards towards the circumference. Another type of circular accelerator, invented in 1940 for accelerating electrons, is the Betatron.
Cyclotrons reach an energy limit because of the relativistic effects at high energies whereby particles become more difficult to accelerate. Though the special theory of relativity precludes matter from traveling faster than the speed of light in a vacuum, the particles in an accelerator normally travel very close to the speed of light. In high-energy accelerators, there is a diminishing return in speed as the particle approaches the speed of light. Therefore particle physicists do not generally think in terms of speed, but rather in terms of a particle's energy, usually measured in electron volts (eV), instead.
Cyclotrons can no longer accelerate protons when they have reached an energy of about 10 million electron volts (10 MeV), because the protons get out of phase with the driving electric field. They continue to spiral outward to a larger radius but, as explained above, no longer gain enough speed to complete the larger circle as quickly. They are nevertheless useful for "lower energy" applications. There are ways for compensating for this to some extentânamely the synchrocyclotron and the isochronous cyclotron.
To make the energies even higher, to billions of electron volts (GeV), it is necessary to use a synchrotron. This is an accelerator in which the particles are contained in a donut-shaped tube, called a storage ring. The tube has many magnets distributed around it to focus the particles and curve their tracks around the tube, and microwave cavities similarly distributed to accelerate them.
The size of Lawrence's first cyclotron was a mere 4 inches (100 mm) in diameter. Fermilab has a ring with a beam path of 4 miles (6 km). The largest circular accelerator ever built was the LEP synchrotron at CERN, with a circumference 26.6 kilometers, which was an electron/positron collider. It has been dismantled and the underground tunnel is being reused for a proton/proton collider called the LHC. The aborted Superconducting Supercollider (SSC) in Texas would have had a circumference of 87 km. Construction was started but it was subsequently abandoned well before completion. Very large circular accelerators are invariably built in underground tunnels a few meters wide to minimize the disruption and cost of building such a structure on the surface, and to provide shielding against the intense synchrotron radiation.
Current accelerators such as the Spallation Neutron Source, incorporate superconducting cryomodules. The Relativistic Heavy Ion Collider, and upcoming Large Hadron Collider also make use of superconducting magnets and RF cavity resonators to accelerate particles.
Targets and detectors
The output of a particle accelerator can generally be directed towards multiple lines of experiments, one at a given time, by means of a deviating electromagnet. This makes it possible to operate multiple experiments without needing to move things around or shutting down the entire accelerator beam. Except for synchrotron radiation sources, the purpose of an accelerator is to generate high-energy particles for interaction with matter.
This is usually a fixed target, such as the phosphor coating on the back of the screen (in the case of a television tube); a piece of uranium in an accelerator designed as a neutron source; or a tungsten target for an X-ray generator. In a linac, the target is simply fitted to the end of the accelerator. The particle track in a cyclotron is a spiral outwards from the center of the circular machine, so the accelerated particles emerge from a fixed point, just as in a linear accelerator.
For synchrotrons, the situation is more complex. Particles are accelerated to the desired energy. Then, a fast-acting dipole magnet is used to switch the particles out of the circular synchrotron tube and towards the target.
A variation commonly used for particle physics research is a collider, also called a "storage ring collider." Two circular synchrotrons are built in close proximityâusually on top of each other and using the same magnets (which are then of more complicated design to accommodate both beam tubes). Bunches of particles travel in opposite directions around the two accelerators and collide at intersections between them. This can increase the energy enormously; whereas in a fixed-target experiment the energy available to produce new particles is proportional to the square root of the beam energy, in a collider the available energy is linear.
Higher energies
At present, the highest energy accelerators are all circular colliders, but it is likely that limits have been reached in respect of compensating for synchrotron radiation losses for electron accelerators, and the next generation will probably be linear accelerators 10 times the current length. An example of such a next generation electron accelerator is the 40 km long International Linear Collider, due to be constructed between 2015-2020.
As of 2005, it is believed that plasma wakefield acceleration in the form of electron-beam "afterburners" and standalone laser pulsers will provide dramatic increases in efficiency within two to three decades. In plasma wakefield accelerators, the beam cavity is filled with a plasma (rather than vacuum). A short pulse of electrons or laser light either constitutes or immediately trails the particles that are being accelerated. The pulse disrupts the plasma, causing the charged particles in the plasma to integrate into and move toward the rear of the bunch of particles that are being accelerated. This process transfers energy to the particle bunch, accelerating it further, and continues as long as the pulse is coherent.[1]
Energy gradients as steep as 200 GeV/m have been achieved over millimeter-scale distances using laser pulsers[2] and gradients approaching 1 GeV/m are being produced on the multi-centimeter-scale with electron-beam systems, in contrast to a limit of about 0.1 GeV/m for radio-frequency acceleration alone. Existing electron accelerators such as SLAC could use electron-beam afterburners to greatly increase the energy of their particle beams, at the cost of beam intensity. Electron systems in general can provide tightly collimated, reliable beams; laser systems may offer more power and compactness. Thus, plasma wakefield accelerators could be usedâif technical issues can be resolvedâto both increase the maximum energy of the largest accelerators and to bring high energies into university laboratories and medical centers.
Black hole production
In the next few decades, the possibility of black hole production at the highest energy accelerators may arise, if certain predictions of superstring theory are accurate.[3] If they are produced, it is thought that black holes would evaporate extremely quickly via Hawking radiation. However, the existence of Hawking radiation is controversial.[4] It is also thought that an analogy between colliders and cosmic rays demonstrates collider safety. If colliders can produce black holes, cosmic rays (and particularly ultra-high-energy cosmic rays) should have been producing them for eons, and they have yet to harm earth.
Notes
- â Matthew Wright and Early Wright, Riding the Plasma Wave of the Future. Symmetry: Dimensions of Particle Physics (Fermilab/SLAC).
- â B.N. Briezman, et al, Self-Focused Particle Beam Drivers for Plasma Wakefield Accelerators. Retrieved October 9, 2007.
- â ESI Special Topics, An Interview with Dr. Steve Giddings. Retrieved October 9, 2007.
- â Adam D. Helfer, Do black holes radiate? Rept. Prog. Phys. 66:943. Retrieved October 9, 2007.
ReferencesISBN links support NWE through referral fees
- Wiedemann, Helmut. 2007. Particle Accelerator Physics. New York: Springer. ISBN 3540490434
- Wille, Klaus and Jason McFall. 2001. The Physics of Particle Accelerators: An Introduction. New York: Oxford University Press. ISBN 0198505493
- Wilson, E.J.N. 2001. An Introduction to Particle Accelerators. New York: Oxford University Press. ISBN 0198508298
External links
All links retrieved November 18, 2022.
- Particle accelerator research
- Particle Accelerators around the world.
- Panofsky, Wolfgang K.H. 1997. The Evolution of Particle Accelerators & Colliders. Stanford.
- Kestenbaum, David. 2007. Massive Particle Accelerator Revving Up. NPR.
- RTFTechnologies.org Electrostatic Particle Accelerator.
Credits
New World Encyclopedia writers and editors rewrote and completed the Wikipedia article in accordance with New World Encyclopedia standards. This article abides by terms of the Creative Commons CC-by-sa 3.0 License (CC-by-sa), which may be used and disseminated with proper attribution. Credit is due under the terms of this license that can reference both the New World Encyclopedia contributors and the selfless volunteer contributors of the Wikimedia Foundation. To cite this article click here for a list of acceptable citing formats.The history of earlier contributions by wikipedians is accessible to researchers here:
The history of this article since it was imported to New World Encyclopedia:
Note: Some restrictions may apply to use of individual images which are separately licensed.